Making and Breaking Connections in the Brain
- Published18 Nov 2020
- Author Amber Dance
- Source Knowable Magazine
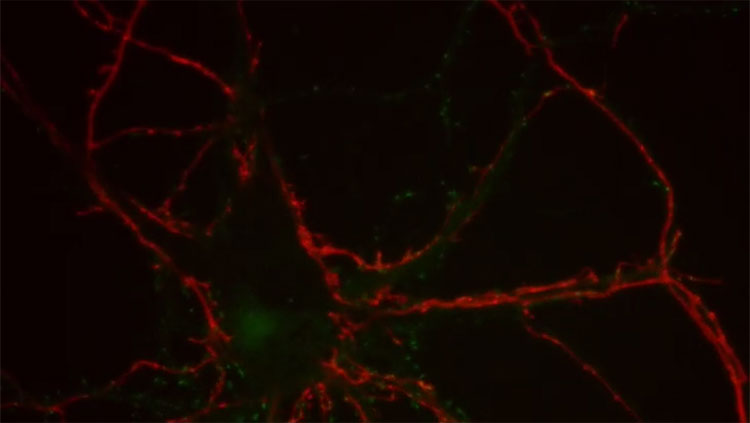
If you were to take a human brain and toss it in a blender — not that you should — the resulting slurry of cells wouldn’t be special in the way that the human brain is. No thoughts, no worries, no wonder or awe.
That’s because it’s the connections between those cells that make the brain so amazing. By sending electrical signals from nerve cell to nerve cell within a great network of connections, the brain creates thoughts as mundane as “Where are my keys?” or as profound as “I think, therefore I am.”
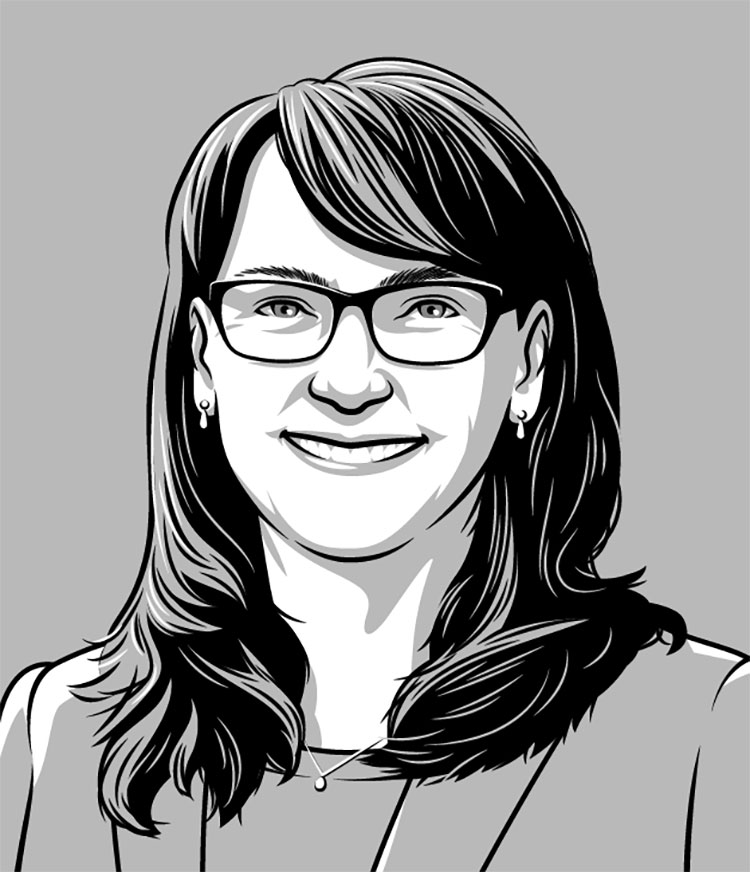
Kimberley McAllister has been fascinated by the human brain since college. As a graduate student in the 1990s studying developmental neurobiology, she was drawn to the question of how the brain is built: how individual brain cells in a growing fetus somehow organize themselves into an organ capable one day of pondering the mysteries of life.
Now director of the Center for Neuroscience at the University of California, Davis, McAllister continues to investigate how the brain’s nerve cells — called neurons — find each other, connect and disengage. She spoke with Knowable Magazine about key discoveries in the study of brain networks, and new work revealing their importance in disease.
This conversation has been edited for length and clarity.
The links between neurons are called synapses. What exactly is a synapse, and what happens there?
It’s basically a connection: one cell talking to another. A brain cell, or a neuron, has a large main body, with small strands sticking out. So one neuron, the transmitter, uses a really thin strand called an axon. A second neuron, the receiver, can receive contacts along its main body, or along strands that branch out like a tree, called dendrites. When the axon tip of a transmitter connects to a receiver, that’s a synapse.
Neurons run on electricity. If an electrical signal passes down an axon, its tip releases chemicals called neurotransmitters into the synapse. These neurotransmitters tell the receiver cell to either activate its own electrical charge, which sends the signal to the next neuron in the chain, or tell the receiver cell to stay quiet.
But it’s not as simple as one receiver for every transmitter. For example, in the frontal cortex — the part of the brain housing abilities such as language that distinguish us from other animals — the neurons look beautiful, like trees. They can have 10,000 or more synapses on their branching dendrites, each of which may receive information from a different cell.
The activity at those thousands of inputs gets added up to cause the neuron to fire — or not — and that’s how information is transferred in the brain. This kind of information transfer, across complicated networks made by the 120 billion neurons in the human brain, allows for complex thoughts.
Axons and dendrites can move around, especially when the brain is young. The way in which they connect individual neurons creates the network pathways. During development, the 100 trillion synapses in the human cortex form at a rate of an estimated 10,000 every 15 minutes! Together, all these synapses create a giant network. And that gives us consciousness.
You’ve been studying synapses for more than two decades. How has our understanding evolved?
When I started, we didn’t know anything about how synapses form. I developed a technique to study dendrite growth. What we realized is that the shape of neurons and dendrites is dependent on activity at synapses. That is, if the brain isn’t getting input — from the senses and the environment — and responding with conversations across the network, then the neurons won’t build the right receivers, and the brain won’t develop properly.
Synapses are very small but incredibly complex molecular machines made up of proteins that guide, maintain and strengthen the connections. One of the biggest advances, over the last 20 years, has been our identification of the tremendous number of proteins that make up these connections. Biochemists estimate there are thousands of different, distinct proteins at each synapse. The amazing diversity of these proteins is what allows the brain to tune the strength and stability of synapses, allowing us to think complex thoughts and build memories.
We’ve learned that if there is a genetic mutation that changes the function of one of these proteins, it can contribute to disorders such as autism, schizophrenia and depression. We’ve come to think of these conditions as synapse disorders, or synaptopathies.
In a developing fetus or a baby, neurons have been thought to form lots of connections willy-nilly, as described by the delightful phrase “exuberant synaptogenesis.” How does this process build an organized brain?
When neurons are born in the fetal brain, they migrate to their proper position. A simplified way of thinking about it is that some move to the cerebrum which is involved in jobs like speech and reasoning, some to the cerebellum, which is involved in coordinating movement, and some to the brain stem where automatic body actions like breathing are rooted. Once positioned, the axons then follow chemical trails to target areas, either in the same part of the brain or elsewhere.
In textbooks, it says that once they are in the basic target area, axons form exuberant connections; then the excess synapses are pruned only later in development. But we now know there are molecules that limit synapse formation to start with, that the initial formation is more tightly controlled.
The ability of the brain to strengthen or weaken synapses depending on how active they are is often referred to as “plasticity.” What is plasticity and why is it important?
Plasticity means the brain can change, for example by altering connections in its networks. Without plasticity, we wouldn’t be able to learn or adapt to our environment. When you learn something, you have electrical activity going through different circuits. Those electrical impulses change the strength of specific connections, making them either stronger or weaker.
For example, if you learn that “hola” means “hello” in Spanish, certain synapses will become stronger. This results from changes in the various proteins making up the synapse.
Scientists can see this when they mimic learning in slices of brain in lab dishes, and even in living animals. Dendrites have little bumps, called spines, that act as signal receivers. After learning, these spines get larger, and they are more likely to stick around. That kind of change is part of plasticity.
Little kids are great at absorbing new information and skills. As adults, we’re often not so good at this. How does plasticity change with age?
The brains of young animals have a lot of plasticity. For each ability in the brain, such as picking up a language, there’s a critical period in which learning is easy. That critical period is when the brain is making lots of changes. If you look at those dendrite spines in a young brain, they’re moving around like crazy.
But when you look in an adult brain, the spines don’t move very much. That’s because material that acts like glue comes in and holds the neurons in place. The critical period for each brain region closes at a different time. For example, the critical period for language development starts to close around age 5. But the brain’s ability to make rational judgments isn’t fully mature until about age 25.
So the connections are pretty stable in adults, but plasticity doesn’t completely disappear. In adults, it’s not so much about adding or deleting connections, but tuning the strength of synapses, using all those synapse proteins.
One of the great mysteries is why adults lose that ability to learn so easily. In some cases, like parts of the visual system in frogs and goldfish, this plasticity doesn’t disappear. Scientists are trying to understand what happens in those creatures. If we could reopen the critical period in someone whose nerves are damaged or deteriorating, maybe we could regrow connections.
To study synapses, scientists first tackled the neuromuscular junction, where certain neurons meet up with muscles to control movement. Where did researchers go from there?
In the 1990s and early 2000s, the field was completely dominated by neuromuscular junction studies. It’s a much simpler synapse than those in the brain. We learned a lot.
But in the brain, synapses are much more diverse. Focusing on the brain became possible with the development of techniques that allowed us to take neurons out of the brain and watch, in a dish, as they form networks. So we can start to assess how synapses form, function and disappear in the complex networks they form even outside of the brain.
What we’ve discovered is there are huge differences between the brain and the neuromuscular junction. There are many, many more diverse types of synapses in the brain, and more kinds of neurotransmitters. It makes the brain much more complicated — not to diminish the complexity of the neuromuscular junction! — and super-interesting.
Researchers have also discovered a new class of molecules that hold the two sides of the synapse together. Why are these important?
These molecules span the gap between the two sides of the synapse, holding the transmitting and receiving cells together like zippers, and they’re really important for synapse formation and elimination.
It turns out that mutations that alter many of these “zipper” molecules make synapses dysfunctional, and are associated with brain disorders including epilepsy, Down syndrome and Alzheimer’s disease. For example, defects in the gene for a zipper protein called neuroligin were linked to autism; then researchers found mutations in the same gene in people with schizophrenia.
But you also can have these mutations and no brain disorder. One of the burning questions right now is, what causes somebody with a zipper protein mutation to manifest symptoms and why do distinct sets of symptoms (i.e. autism vs. schizophrenia) show up in different people with the same mutations?
I think these zipper proteins may turn out to be even more interesting than other parts of the synapse. Scientists now want to understand: How can we develop drugs to fix the dysfunctional synapses?
The brain has been thought to be somewhat isolated from the body’s immune system. Yet you and others are now studying the role of immune cells and molecules in the brain. What do they do?
There is tremendous excitement about a particular kind of immune cell called microglia. These cells clear away dead cells and other used-up materials in the brain. Microglia can eliminate synapses by eating them. We know they’re involved in brain diseases such as Alzheimer’s, because they are switched to their active, anti-infection state in people with those conditions. But it’s still a puzzle exactly how they contribute.
I’ve been studying brain functions of immune molecules called MHC1. These molecules stick off of the surfaces of almost all cells. Their traditional job is to tell the immune system that those cells are part of the body, and not something to attack. MHC1 molecules are also involved in synaptic plasticity. They prevent synapse formation early on, so they make brain development less exuberant and more tightly controlled.
I also think MHC1 molecules are involved in how chemicals or infections affect brain development. I’m part of a group studying how infections in pregnant women exacerbate the baby’s risk for eventual autism spectrum disorder or schizophrenia. In one project, we’re studying the offspring of mother mice after the mothers were exposed to a virus-like substance that provokes an immune response. The offspring mice have more MHC1, and fewer synapses, in their brains than control animals. This suggests that infections that engage the immune system can change brain circuitry through changing levels of immune molecules at synapses.
On the one hand, the fact that infections can alter brain development is scary. On the other hand, most of the time infections don’t affect the brain. If we can better understand how, when and why the immune system regulates brain development, we might one day be able to develop medicines to change the immune response and fix what’s going on, or going wrong, in the brain.
CONTENT PROVIDED BY
Knowable Magazine is an independent journalistic endeavor from Annual Reviews.